First Cable Model of Unmyelinated Vagus Nerve Helps Develop Innovative Treatments
In a study published in 2020, Duke biomedical engineer Dr. Nikki Pelot and her team built upon previous models to create a groundbreaking computational model of an unmyelinated axon, a type of nerve cell specific to the peripheral nervous system that controls the motor system and voluntary functions. This work has helped develop new treatments for neurological diseases that are primarily caused by the degeneration of motor functions such as Alzheimer’s and Parkinson’s. These treatments include drugs—such as blood pressure medications, anesthetics, and sedatives—that target ion channels and create therapeutic electrical stimulation treatments.
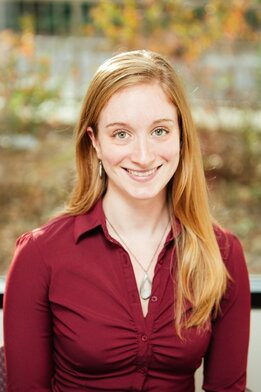
Above: Dr. Nikki Pelot. Image courtesy of nikkipelot.com.
Computational models of axons use mathematics and coding to represent the electrical and mechanical properties of axons. They are valuable for simulating the effects of drugs and electric shock therapies on the vagus nerve. Axons are long extensions of neurons in the brain that transmit electrical signals to other neurons or areas of the body. Many past models focus on modeling axons in the autonomic nervous system, which control involuntary functions of the body, rather than peripheral axons, which affect the motor system and voluntary functions. However, understanding the peripheral nervous system is critical for the creation of treatments for diseases like Parkinson’s that globally impact 8.5 million people each year. Despite the prevalence of these neurological diseases, very few unmyelinated axon models exist. Unmyelinated axons are important since they are the most common type of axon in the peripheral nervous system.
Dr. Pelot and her team studied unmyelinated axons and developed a model of the vagus nerve, a vital nerve located next to the carotid artery that sends signals from brain regions associated with mood and motor functions. Electrical stimulation treatments of the vagus nerve can assist with neurological conditions such as depression or epilepsy.
Myelination is akin to insulation surrounding the axon like the rubber on the outside of a wire, allowing the propagation of electrical and mechanical signals to occur faster along the length of the axon. Within the peripheral nervous system, slower signaling is common from unmyelinated axons as it is more energy-efficient to have unmyelinated neurons send signals over short distances. Faster signals are reserved for urgent input so the brain can prioritize life-preserving functions over less urgent ones.
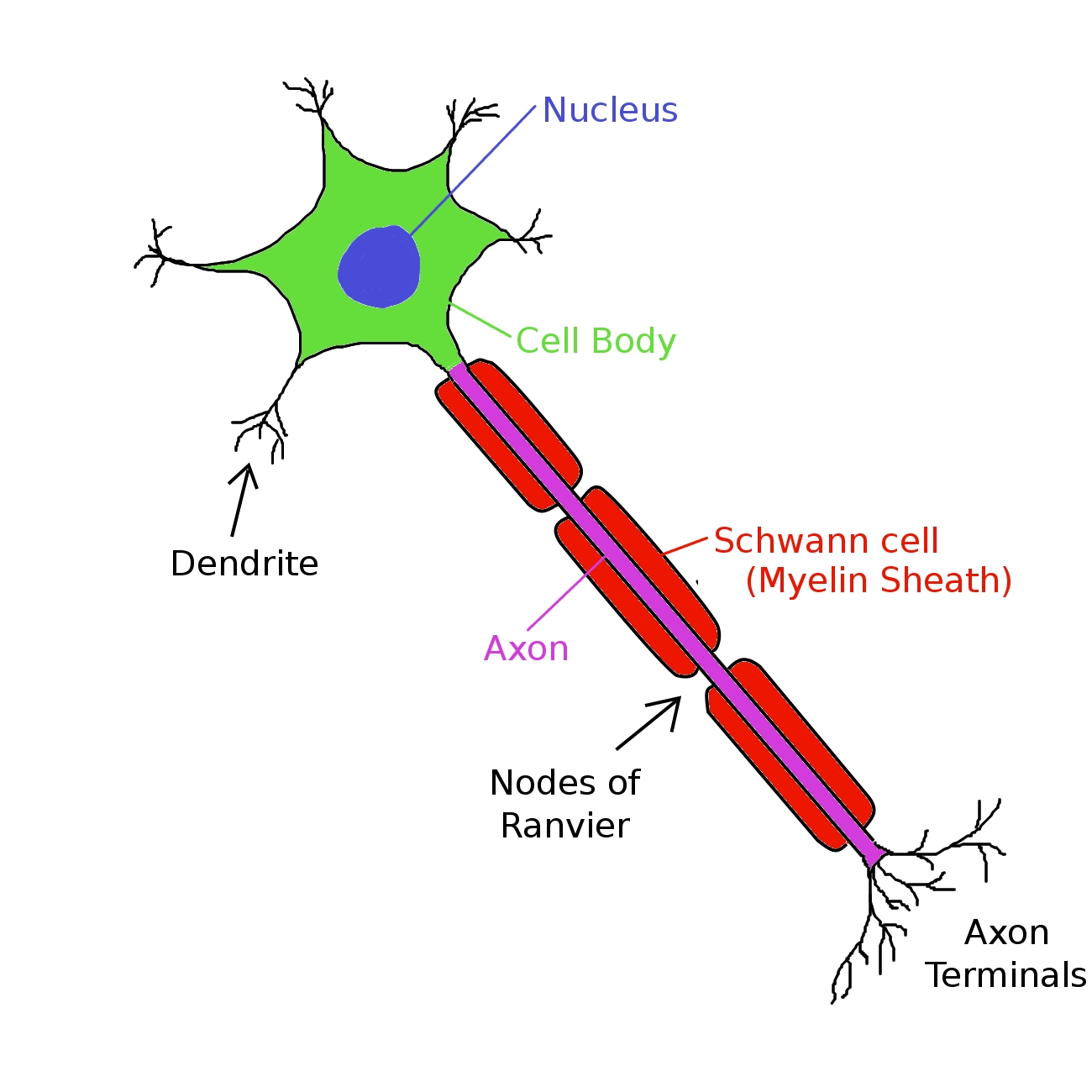
Above: Diagram of a myelinated neuron. Image courtesy of WikiMedia.
To create their model, Dr. Pelot’s team analyzed five published computational models: a basic model of both myelinated and unmyelinated axons, its updated version, and three models focused specifically on unmyelinated axons. They focused on recreating these models while integrating new information and discoveries to develop the first cable model (a geometrically complex representation) of an unmyelinated vagus nerve fiber. Key components they incorporated into their models include ion channel dynamics and conduction velocities, which help make the model more accurate overall.
For example, when the team recreated the simpler single-compartment model, they incorporated ionic conductances to control ion flow throughout the neurons. Ions like sodium, potassium, and calcium affect the flow of electricity through the axon. By adding new equations and information, they transformed the model from a single-compartment approach—which treats the axon as one simple cylinder—into a multi-compartment model that divides the axon into multiple segments—a more accurate representation of axons in the brain.
Through this process, they discovered some discrepancies in past research records. To validate the accuracy of their model, Dr. Pelot collected primary data from single-fiber recordings, which measure electrical signals from individual nerve fibers. They compared this data with their model’s predictions, analyzing resting potential (the electrical charge difference of the neuron’s membrane when it is not transmitting a signal), action potential shape (a graph showing how the voltage inside the neuron changes over time), strength-duration response (the relationship between the voltage of the electrical stimulus and how long it takes to cause a response), and threshold recovery cycle (the neuron’s reduced responsiveness after an action potential). To further ensure the accuracy of their model, they implemented past models into three different software programs. With these checks, they could confirm that observed discrepancies were due to errors in earlier works rather than their own model.

Above: Membrane potential of a neuron during an action potential. Image courtesy of Wikimedia.
A significant finding from comparing their new model with models recreated from past research is that changing the maximum conductances (the peak ability for ions to flow into the neuron) has a greater impact on the electrical properties of the axon than changing the behavior of sodium channels, which gate entry of sodium ions into the neuron.
Dr. Pelot and her team faced many challenges in creating their model, underscoring the need for more research and data to further advance the modeling of autonomic nerves. Thus, their groundbreaking computational model of autonomic axons of the vagus nerve is a monumental step in the development of treatments for nervous system disorders.